Back to the future
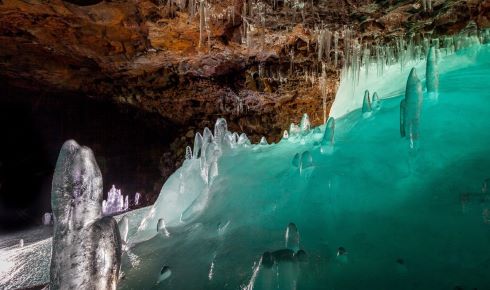
6th September 2024
Could ancient DNA, surviving in permafrost from hot periods in the past, help us save crops from drought and extreme heat in our ever-warming world?
Anthropogenic atmospheric warming is resulting in major disturbances of the global weather systems, with more severe storms and greater rainfall in some regions and long-term droughts in others. Drought causes loss of crop yield or, in the most extreme cases, total loss of the crop. Drought is commonly tackled by irrigation, but this is not always possible, and prolonged irrigation with soil water can lead to increased salinity, which inhibits plant growth.
Microorganisms can be beneficial to plants under stress in various ways: stimulating root growth, producing moisturising compounds and protecting agents, and increasing nutrient availability via extracellular enzymes. One of the newest strategies scientists are investigating is engineering the soil microbiome or, more specifically the rhizosphere microbiome, the microorganisms closely associated with plant roots. The properties of the rhizosphere are critical because all the water transpired by the plant has to go through this thin zone in the soil where the roots, soil and microbes interact. By selectively modifying the composition of the soil flora, bacteria, fungi, and other microorganisms, scientists aim to enhance plant resistance to environmental stressors such as drought.
However, despite promising outcomes observed in the laboratory, attempts to engineer the soil microbiome have yet to be widely adopted in the field. A deeper understanding of plant-microbe evolution could be a catalyst for designing effective strategies.
Old answers to new questions
In the fairly recent period of geological history – i.e. the last million years or so – there have been periods when global temperatures rose naturally to the levels we may reach in the next few decades (about 2°C above the baseline level). Warm climates occurred, for example, in the Holocene Thermal Maximum (around 8,000 years before the present) and the Eemian Interglacial (around 130,000–115,000 years before the present). These natural fluctuations in temperature happened relatively slowly, in contrast to our current temperature increases, which have taken place in a matter of decades and are increasing in rate – the so-called Great Acceleration.
We can assume that in the past, the soil microbiome had a long period to adapt and evolve to these changing conditions – longer than it has to evolve at the present time. The obvious conclusion, therefore, is that if we wish to find soil microorganisms adapted to climate change, we should look to the past. If we could go back in time, we could identify the microbes that naturally evolved to benefit plant adaptation. Thanks to our ability to recover and decode ancient environmental DNA, we can now do this.
DNA can be preserved in nature for up to two million years. Cold and anoxic conditions, such as those found in permafrost, create an ideal environment for preservation. After sampling, ancient permafrost is transported to a dedicated palaeogenetic laboratory and DNA is extracted and processed under the highest standards of cleanliness to avoid contamination with modern DNA.
High-throughput sequencing technologies are then used to retrieve the genetic fingerprint of the entire ecosystem sample, including flora, fauna and microorganisms. Multiple samples from different ages allow the creation of permafrost ‘chronosequences’ – genetic time capsules that enable scientists to reconstruct how past ecosystems responded to climatic changes over time, from the past to the present day.
This is the framework that we are adopting in our project, known as TOLERATE: Adaptation to climate change in the rhizosphere across the millennia. We start from a unique chronosequence of one of the oldest permafrosts in the Arctic, dating back around 700,000 years and covering several cycles of temperature fluctuations.
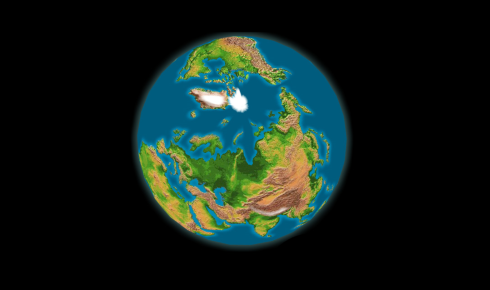
The Arctic region warms faster than the rest of the planet – a phenomenon known as Arctic amplification – and has experienced significant temperature swings during the past glacial and interglacial periods, making it a critical area for studying climate change and predicting future climate scenarios.
A stitch in time
While palaeoclimatologists reconstruct the approximate chronology and climate conditions, the palaeogenetic laboratory extracts DNA and performs sequencing using the so-called shotgun metagenomics approach. This involves untargeted sequencing of all DNA fragments present within a given environmental sample, capturing all the microorganisms, plants and animals together, providing insights into their genetic composition, biodiversity and functional capabilities.
This approach is relatively well established for the analysis of modern communities, but its application to ancient DNA is less straightforward. Ancient DNA is highly degraded due to years of chemical processes such as hydrolysis, oxidation and depurination acting on the matter. The cumulative effect is shorter, fragmented strands with damaged nucleotide sequences, making extraction and analysis challenging and requiring specialised techniques.
The fragments in most of our samples are less than 100 basepairs, significantly shorter when compared with a full bacterial genome which may be two to five million basepairs. Scientists have demonstrated that single-stranded DNA library preparation yields significantly better outcomes for ancient DNA compared with double-stranded DNA library preparation.
Processing ancient DNA also involves stringent contamination control measures, such as using dedicated clean rooms, full-body protective clothing and UV irradiation, along with extensive use of negative controls. To authenticate ancient DNA, we look at the frequency of cytosine to thymine transitions, a common damage pattern caused by cytosine deamination over time. This ultimately confirms that the DNA sequence is genuinely ancient and free from modern contamination.
Other bioinformatic tools are used to stitch these short fragments together and reassemble them into contigs of longer length, allowing us to obtain complete genes and even estimate entire genomes, so-called metagenome-assembled genomes.
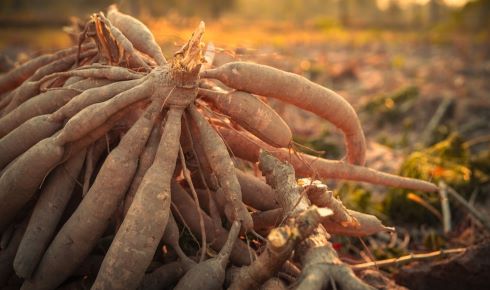
Extracting data
The first useful data we can extract from the samples is the overall composition of the microbiome, i.e. the identity of all microorganisms present in the soil environment. We can use this information to reconstruct the rhizosphere microbiome, over millennia, following how it changed in response to colder and warmer climates, giving us insights into which taxa likely played (or play) an important role in plant adaptation. We can also zoom in on the genetic information relating to particularly relevant functions. These include ‘osmoprotectant’ compounds that help the plant retain water, extracellular polysaccharides, which modify the properties of the soil, heat shock proteins, which help protect other proteins under heat stress, and extremozymes, enzymes capable of working under extreme conditions.
The challenge is then how to harness the capabilities of these ancient organisms and their functionalities when they are no longer viable and cannot be cultivated in the laboratory. Our strategy is to integrate palaeo-metagenomics with synthetic biology. Once we have identified promising genes from the ancient DNA metagenomes, the next step is to express them in suitable platform organisms. These are non-pathogenic, soil inhabiting bacteria that we can use as bioaugmentation vehicles for the rhizosphere of modern day crops. The top candidate is Pseudomonas putida, a soil bacterium and a well-established industrial chassis with a history of being used in this context.
Once cloned host organisms have been generated and tested in the laboratory, larger quantities will be fermented and freeze-dried for storage and transport. Upon re-activation, these organisms will be used as soil bioinoculants and assessed for their beneficial effects on the growth of crop plants, such as barley, under drought conditions. Here, it will be crucial to evaluate bacterial survival and population density, and to determine the shelf life of the freeze-dried state. Both these factors are important for a commercially viable product.
A major part of our study will be assessing the ability of the bioinoculants to survive and establish in their new environment, and of course the extent to which they can improve plant growth. Initial experiments will be conducted under controlled laboratory conditions, with further experiments under field conditions dependent on government legislation.
This and other similar approaches to mitigate the effects of climate change appear promising, but they are still encountering significant regulatory challenges. The EU’s current genetically modified organism (GMO) legal framework, established in 2001, strictly regulates the introduction of DNA from other species into animals and plants. This framework, driven by a risk-based and precautionary approach, raises concerns about potential impacts on human health, biodiversity, and the preservation of flora and fauna. Similarly stringent rules apply to genetically modified microorganisms.
This complex regulatory environment poses barriers to the development and use of innovative genetic modification techniques. Under the GMO Release Directive, GMOs must undergo an authorisation procedure before being marketed and cultivated in the EU. Owing to the relentless progress of technological development in the agricultural sector, the current GMO legal framework is no longer fit for purpose and requires adaptation to scientific advancements.
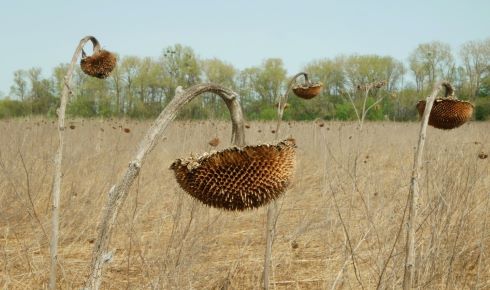
A change in framework
To complicate matters further, since Brexit the UK has signalled its intention to adopt a more permissive approach to gene editing than that of the EU. This reflects a shift towards a more science-driven and innovation-friendly legal framework, particularly for precision-bred organisms.
The new UK legislation aims to facilitate gene editing without requiring the complex approvals mandated under the existing EU GMO regime. However, regarding the regulation of conventional GMOs in UK, EU rules still apply.
An evolving political approach is paving the way for new regulatory frameworks. The TOLERATE project will also scrutinise the GMO and new genomic techniques regulatory framework to identify hindrances and potentials in the development, authorisation and use of engineered rhizosphere bacteria, considering current and forthcoming legal and policy changes.
Our hope is that our research can lead to new agricultural products that use the innovation of ancient plants and microbes to help modern-day crops adapt to a rapidly changing world.
Dr Amedea Perfumo is a research scientist at the Alfred Wegener Institute, Helmholtz Centre for Polar and Marine Research, Germany.
Dr Roger Marchant FRSB is professor of biological sciences at Ulster University.
Dr Mirta Alessandrini is a senior lecturer and researcher in agri-food law at the Wageningen University and Research, The Netherlands.